Intro to Oligonucleotide Analysis
Navigation |
---|
I. Oligonucleotide Analysis |
II. Oligonucleotide Synthesis |
III. Oligonucleotide-based Therapeutics |
IV. Oligonucleotide Quality Control Techniques |
I. Oligonucleotide Analysis
Synthetic oligonucleotides have been widely used in various biochemical research areas, such as primers for polymerase chain reaction (PCR), recently synthetic oligonucleotides have become an area of increased interest with the introduction of mRNA based vaccines and other oligonucleotide based therapeutics.1–4 These therapeutics can be designed to specifically target mRNAs, miRNAs, and proteins of various diseases.5–7 The use of oligonucleotide-based therapeutics has increased drastically over the last decade.8,9 This increase has been due to the advances to the speed and ease of synthesis.10,11 Increased synthetic demand comes with it the need for robust analysis techniques to transition synthetic oligonucleotides towards the world of biomedicines.
Oligonucleotides are nucleic acid polymers comprised of either deoxyribonucleic acid (DNA) or ribonucleic acid (RNA) bases.12 The nucleosides of DNA had been thoroughly investigated to understand that there were two groups: purine, and pyrimidines.13–16 These were distinguished by the characteristics of the nucleobase. The purine bases (adenosine and guanine) are two-carbon nitrogen ring bases where the pyrimidines (cytosine, thymine, and uracil (RNA only) ) are one carbon nitrogen ring base (Figure 1).The first structure of DNA was first proposed by Pauling and Corey17, the structure was later investigated through x-ray crystallography to be a helical arrangement of two strands by specific base pair alignments.18 From this work Watson and Crick had proposed that the strands of DNA were paired so that a purine aligned with a pyrimidine; adenine paired with thymine and guanine paired with cytosine. This pairing would allow for sufficient hydrogen bonding to keep the strands together (Figure 2). From that point onward, oligonucleotides had become an item of interest in numerous areas of research, including the development of custom synthetic sequences of both DNA and RNA for use of therapeutics.19,20
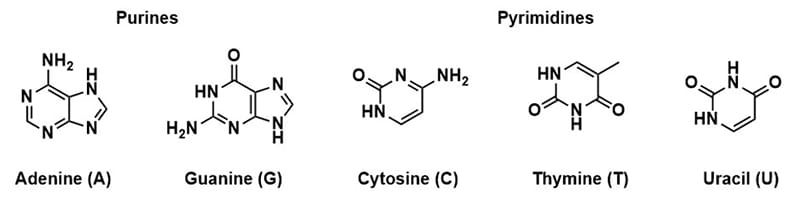
Figure 1. Structure of nucleic acid bases. Purine bases adenosine and guanine, Pyrimidine bases cytosine, thymine, and uracil (RNA only).
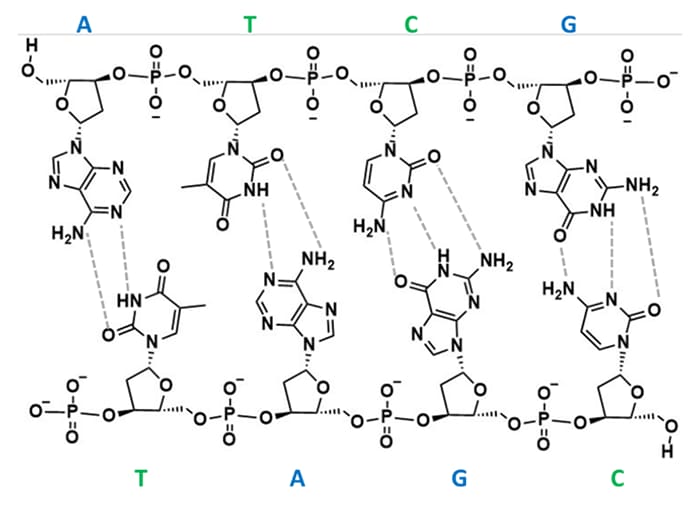
Figure 2. DNA strand showing hydrogen bond patterns of Watson-Crick base pairing.
II. Oligonucleotide Synthesis
Solid phase oligonucleotide synthesis was first introduced by Robert Letsinger in 1964, in this work a polystyrene resin was used as the support for the building of the oligonucleotide.21 This work followed the introduction of peptide synthesis off a resin support. For Letsinger’s synthetic scheme, the bond between nucleotides was formed over a two-step process of phosphorylation of the 3'- hydroxy group with an activated phosphomonoester, followed by coupling a second nucleoside to complete the bond. Letsinger continued to improve this synthetic technique and moved to using a phosphodiester, and ultimately a phosphotriester to simplify synthesis.
Letsinger improved on his original strategy through the development of 3’ and 5’ protected nucleobases. This strategy utilized phosphotriester intermediates that were protected with a β-cyanoethyl ester at the phosphorous and a dimethoxy trityl group at the 5’ hydroxyl (Figure 3). These changes allowed for synthesis automation where the 3’ hydroxyl is attached to the resin support and base coupling occurs between the 5’ hydroxyl of the attached base and an activated 3’ phosphorous of the incoming base.22–25 The work started by Letsinger led to the automation of oligonucleotide synthesis for both DNA and RNA, opening the possibilities of synthesizing custom sequences for various applications (Figure 4).
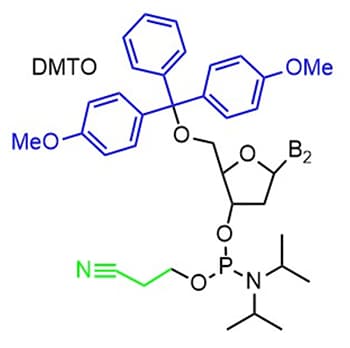
Figure 3. DNA base with 5’ dimethoxy trityl (DMTO) protecting group in blue and 3’ β-cyanoethyl ester protecting group in green.
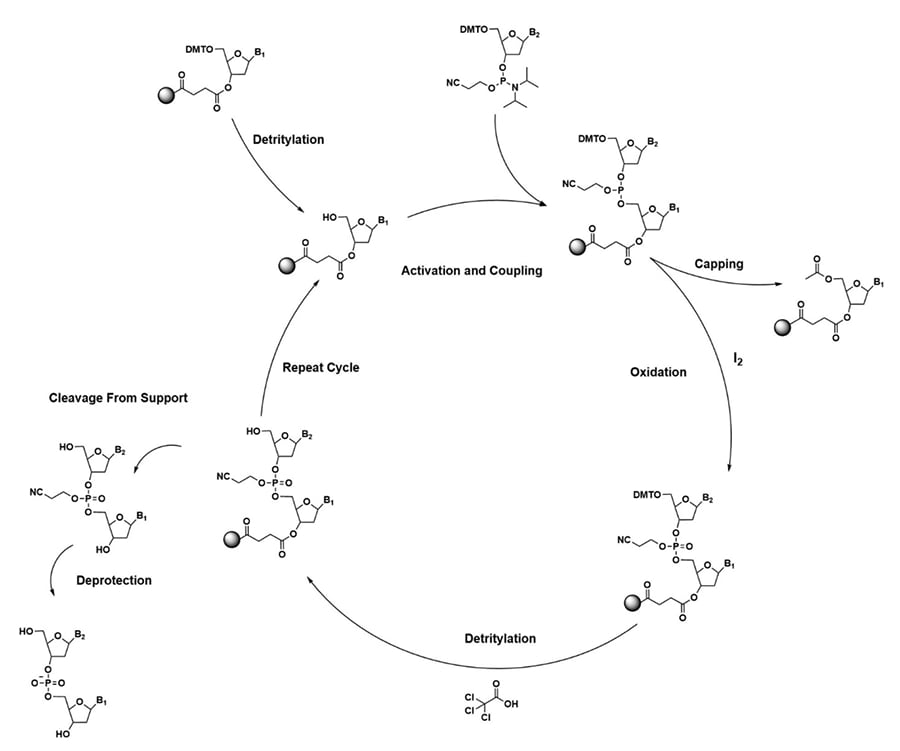
Figure 4. Solid-Phase DNA synthesis cycle using a phosphotriester activated nucleoside.
III. Oligonucleotide-based Therapeutics
Oligonucleotide therapeutics have been utilized to target diseases and disorders that are often associated with undruggable proteins. They usually act through preventing, replacing, adding, or editing RNA or DNA at the molecular level.8 Development of oligonucleotide therapeutics can be based on primary sequence information as these therapeutics act through nucleotide binding according to Watson-Crick base pairing.26 They can be chemically synthesized to give the exact sequence needed for targeting different proteins.
Oligonucleotide-based therapeutics include antisense oligonucleotides27, MicroRNA7, messenger RNA28, small interfering RNA (siRNA)5 and aptamers29. This targeted approach opens the possibility for specified therapeutics for otherwise undruggable diseases and disorders. The increase in interest in oligonucleotide therapeutics is due to several factors; wide range of disease targets, ease and speed of synthesis, and lower manufacturing cost in comparison to small molecule-based therapeutics.6,30,31 There are some limitations when it comes to oligonucleotide-based therapeutics; oligonucleotide instability, inefficient delivery, and off-target effects.9,32,33 Due to the natural susceptibility of oligonucleotides to enzymatic degradation in vivo, chemical modifications have become common to improve stability, target specificity and potency. Some of these modifications include chemical modifications, phosphorothioation (replacement of the phosphorous oxygen bond with a phosphorous sulfur bond) and conjugations with N-acetylgalactosamine (GalNac).3,34
IV. Oligonucleotide Quality Control Techniques
Chemically synthesized oligonucleotides come with the necessity of analyzing the final product and any byproducts from synthesis before moving to in vitro or in vivo testing. There are various techniques that can be utilized to determine oligonucleotide sample purity, molecular weight, and sequence confirmation.
Determining purity of synthesized oligonucleotides can be done through using high performance liquid chromatography (HPLC) to identify different species present.35,36 HPLC utilizes a ultraviolet (UV) detector to monitor the elution of the sample components. Monitoring elution at 254 nm, where the absorption of DNA and RNA is near its maximum.37 This technique allows for separation and detection of other synthesis-based impurities. Utilizing an HPLC that uses biologically inert tubing can prevent loss of signal intensity and lead to more consistent analysis.38,39
The use of HPLC combined with mass spectrometry (LCMS ) or gas chromatography with mass spectrometry (GC/MS ) can also be utilized to identify the composition of the synthetic oligonucleotide.40,41 These techniques can require acid or enzyme hydrolysis prior to analysis.42–45 These additional processing steps increase the overall time for sample analysis. Matrix assisted laser desorption/ionization time-of-flight mass spectrometry (MALDI-TOF MS ) has been utilized for oligonucleotide analysis.46 MALDI-TOF MS can be utilized to determine the mass of the full oligonucleotide along with the sequence through fragmentation analysis.47
MALDI-TOF-MS provides a combination of rapid analysis, high mass resolving power and analyte sensitivity. These key attributes make MALDI a tool well suited for an oligonucleotide synthesis lab, allowing scientists to efficiently determine the oligonucleotide mass and identify the presence of any byproducts. MALDI-TOF MS can be utilized to confirm the sequence of synthesized oligonucleotides through in-source decay (ISD). In this technique, specific MALDI matrices and increased laser power cause a combination of ionization and fragmentation for analysis. Oligonucleotides can fragment at a variety of positions within the phosphate group to yield different classes of ions (Figure 5). From the fragments generated, the sequence of the oligonucleotide can be confirmed through the mass differences of the generated peaks. An example of using MALDI ISD for sequence confirmation is shown in Table 1 and Figure 6. Using MALDI ISD allows for analysis of canonical and non-canonical nucleosides, increasing the range of oligonucleotides that can be analyzed using this method. MALDI-TOF MS can also be utilized to analyze peptide nucleic acids (PNA), compounds that have an amide bond backbone with nucleoside groups rather than amino acids. ( Abban, T. 2017 )
The addition of MALDI-TOF MS to a synthetic routine gives researchers the ability to rapidly identify a wide variety of synthetic products. MALDI-TOF MS fits well in both QC and research and development laboratories.
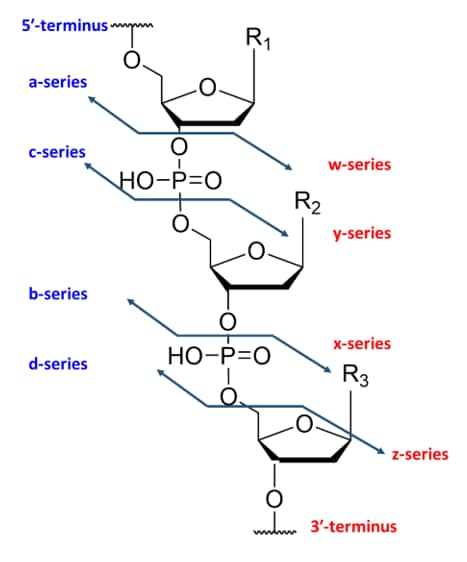
Figure 5. Ion fragmentation nomenclature of oligonucleotides.
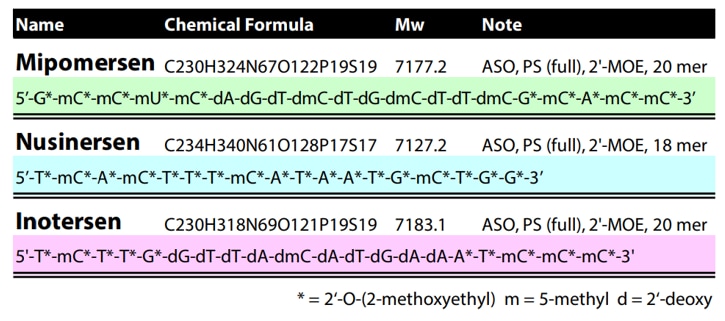
Table 1. Sequences of the model nucleic acids used for MALDI ISD in Figure 6.
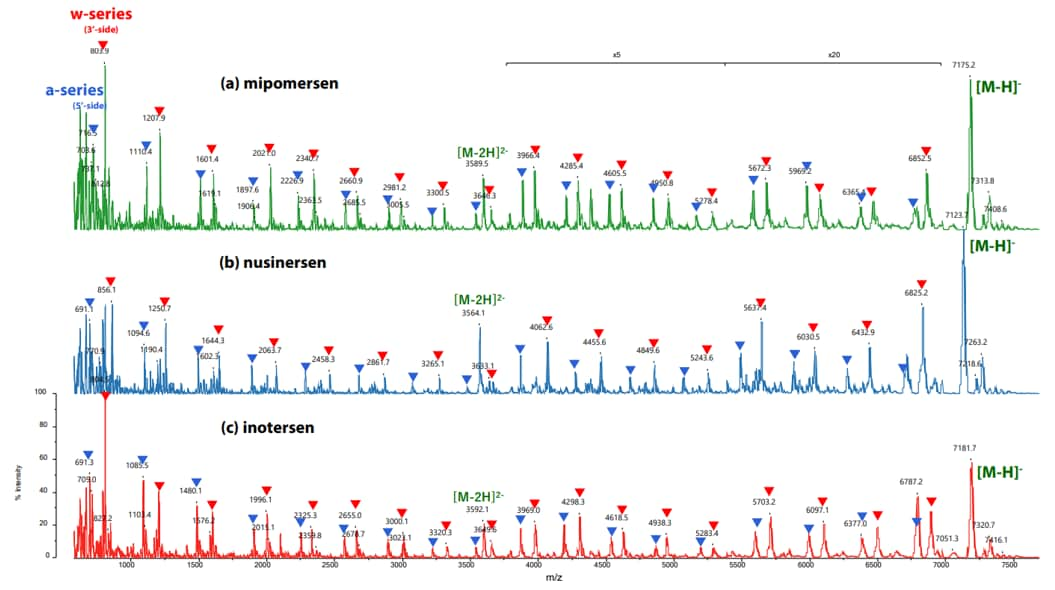
Figure 6. In-Source Decay (ISD) of an oligonucleotide in negative ionization mode using the MALDI-8030. Nucleic acid therapeutics were spotted with HPA as a matrix for analysis (a) mipomersen, (b) nusinersen, and (c) inotersen. A-series ions are marked with blue triangles and W-series ions are marked with red triangles. (Nishikaze, T. 2022 )
[1] Hughes, R. A.; Ellington, A. D. Synthetic DNA Synthesis and Assembly: Putting the Synthetic in Synthetic Biology. Cold Spring Harb Perspect Biol 2017, 9 (1). https://doi.org/10.1101/cshperspect.a023812.
[2] Pardi, N.; Hogan, M. J.; Porter, F. W.; Weissman, D. MRNA Vaccines — a New Era in Vaccinology. Nat Rev Drug Discov 2018, 17 (4), 261–279. https://doi.org/10.1038/nrd.2017.243.
[3] Khvorova, A.; Watts, J. K. The Chemical Evolution of Oligonucleotide Therapies of Clinical Utility. Nat Biotechnol 2017, 35 (3), 238–248. https://doi.org/10.1038/nbt.3765.
[4] Morya, V.; Walia, S.; Mandal, B. B.; Ghoroi, C.; Bhatia, D. Functional DNA Based Hydrogels: Development, Properties and Biological Applications. ACS Biomater Sci Eng 2020, 6 (11), 6021–6035. https://doi.org/10.1021/acsbiomaterials.0c01125.
[5] Hu, B.; Zhong, L.; Weng, Y.; Peng, L.; Huang, Y.; Zhao, Y.; Liang, X.-J. Therapeutic SiRNA: State of the Art. Signal Transduct Target Ther 2020, 5 (1), 101. https://doi.org/10.1038/s41392-020-0207-x.
[6] Burnett, J. C.; Rossi, J. J. RNA-Based Therapeutics: Current Progress and Future Prospects. Chem Biol 2012, 19 (1), 60–71. https://doi.org/10.1016/j.chembiol.2011.12.008.
[7] Rupaimoole, R.; Slack, F. J. MicroRNA Therapeutics: Towards a New Era for the Management of Cancer and Other Diseases. Nat Rev Drug Discov 2017, 16 (3), 203–222. https://doi.org/10.1038/nrd.2016.246.
[8] Thakur, S.; Sinhari, A.; Jain, P.; Jadhav, H. R. A Perspective on Oligonucleotide Therapy: Approaches to Patient Customization. Frontiers in Pharmacology. Frontiers Media S.A. October 19, 2022. https://doi.org/10.3389/fphar.2022.1006304.
[9] Mehta, M.; Deeksha; Tewari, D.; Gupta, G.; Awasthi, R.; Singh, H.; Pandey, P.; Chellappan, D. K.; Wadhwa, R.; Collet, T.; Hansbro, P. M.; Kumar, S. R.; Thangavelu, L.; Negi, P.; Dua, K.; Satija, S. Oligonucleotide Therapy: An Emerging Focus Area for Drug Delivery in Chronic Inflammatory Respiratory Diseases. Chem Biol Interact 2019, 308, 206–215. https://doi.org/10.1016/j.cbi.2019.05.028.
[10] Brown, D. M. A Brief History of Oligonucleotide Synthesis. In Protocols for Oligonucleotides and Analogs; Humana Press: New Jersey; pp 1–18. https://doi.org/10.1385/0-89603-281-7:1.
[11] Song, L.-F.; Deng, Z.-H.; Gong, Z.-Y.; Li, L.-L.; Li, B.-Z. Large-Scale de Novo Oligonucleotide Synthesis for Whole-Genome Synthesis and Data Storage: Challenges and Opportunities. Front Bioeng Biotechnol 2021, 9. https://doi.org/10.3389/fbioe.2021.689797.
[12] Jeremy M. Berg; John L. Tymoczko; Gregory J. Gatto Jr.; Lubert Stryer. Biochemistry, 8th ed.; W.H. Freeman, 2015.
[13] Masson Gulland, B. J. A Lecture Delivered Before The Chemical Society On May 5th, 1938.*; 1938. https://doi.org/10.1101/SQB.1947.012.01.011.
[14] Gulland, J. M.; Story, L. F. 50. Constitution of the Purine Nucleosides. Part VI. Adenine Deoxyriboside, Adenine Glucoside, and a Route to the Synthesis of the Naturally Occurring Nucleosides. Journal of the Chemical Society (Resumed) 1938, 259. https://doi.org/10.1039/jr9380000259.
[15] Masson Gulland, J.; Story, L. F. Guanine Deoxyriboside. The Method of Determining the Position of the Carbohydrate Radical in Purine Nucleo; 1934.
[16] Furberg, S.; Jensen, M. B.; Faurholt, C. On the Structure of Nucleic Acids. Acta Chem Scand 1952, 6, 634–640. https://doi.org/10.3891/acta.chem.scand.06-0634.
[17] Pauling, L.; Corey, R. B. A Proposed Structure For The Nucleic Acids. Proceedings of the National Academy of Sciences 1953, 39 (2), 84–97. https://doi.org/10.1073/pnas.39.2.84.
[18] WATSON, J. D.; CRICK, F. H. C. Molecular Structure of Nucleic Acids: A Structure for Deoxyribose Nucleic Acid. Nature 1953, 171 (4356), 737–738. https://doi.org/10.1038/171737a0.
[19] Ran, F. A.; Hsu, P. D.; Wright, J.; Agarwala, V.; Scott, D. A.; Zhang, F. Genome Engineering Using the CRISPR-Cas9 System. Nat Protoc 2013, 8 (11), 2281–2308. https://doi.org/10.1038/nprot.2013.143.
[20] Glazier, D. A.; Liao, J.; Roberts, B. L.; Li, X.; Yang, K.; Stevens, C. M.; Tang, W. Chemical Synthesis and Biological Application of Modified Oligonucleotides. Bioconjug Chem 2020, 31 (5), 1213–1233. https://doi.org/10.1021/acs.bioconjchem.0c00060.
[21] Merrifield ; R, ) R B; Letsinger, L. L.; Hamilton, S. B. Reactions on Polymer Supports 5163 ORGANIC AND BIOLOGICAL CHEMISTRY Reactions on Polymer Supports1 “Popcorn” Polymer Could Be Obtained Directly by Copo-Analogous Transformations Have Been Carried out on the Other Polymer Systems. Details Are given in The; 1962; Vol. 24. https://pubs.acs.org/sharingguidelines.
[22] Letsinger, R. L.; Mahadevan, V. Oligonucleotide Synthesis on a Polymer Support. J Am Chem Soc 1965, 87 (15), 3526–3527. https://doi.org/10.1021/ja01093a058.
[23] Letsinger, R. L.; Ogilvie, K. K. Convenient Method for Stepwise Synthesis of Oligothymidylate Derivatives in Large-Scale Quantities. J Am Chem Soc 1967, 89 (18), 4801–4803. https://doi.org/10.1021/ja00994a048.
[24] Letsinger, R. L.; Kornet, M. J. Popcorn Polymer as a Support in Multistep Syntheses. J Am Chem Soc 1963, 85 (19), 3045–3046. https://doi.org/10.1021/ja00902a054.
[25] Letsinger, R. L.; Ogilvie, K. K. Nucleotide Chemistry. XIII. Synthesis of Oligothymidylates via Phosphotriester Intermediates. J Am Chem Soc 1969, 91 (12), 3350–3355. https://doi.org/10.1021/ja01040a042.
[26] WATSON, J. D.; CRICK, F. H. C. Molecular Structure of Nucleic Acids: A Structure for Deoxyribose Nucleic Acid. Nature 1953, 171 (4356), 737–738. https://doi.org/10.1038/171737a0.
[27] Dhuri, K.; Bechtold, C.; Quijano, E.; Pham, H.; Gupta, A.; Vikram, A.; Bahal, R. Antisense Oligonucleotides: An Emerging Area in Drug Discovery and Development. J Clin Med 2020, 9 (6), 2004. https://doi.org/10.3390/jcm9062004.
[28] Gote, V.; Bolla, P. K.; Kommineni, N.; Butreddy, A.; Nukala, P. K.; Palakurthi, S. S.; Khan, W. A Comprehensive Review of MRNA Vaccines. Int J Mol Sci 2023, 24 (3), 2700. https://doi.org/10.3390/ijms24032700.
[29] Di Ruscio, A.; de Franciscis, V. Minding the Gap: Unlocking the Therapeutic Potential of Aptamers and Making up for Lost Time. Mol Ther Nucleic Acids 2022, 29, 384–386. https://doi.org/10.1016/j.omtn.2022.07.012.
[30] Wadhwa, A.; Aljabbari, A.; Lokras, A.; Foged, C.; Thakur, A. Opportunities and Challenges in the Delivery of MRNA-Based Vaccines. Pharmaceutics 2020, 12 (2), 102. https://doi.org/10.3390/pharmaceutics12020102.
[31] Kher, G.; Trehan, S.; Misra, A. Antisense Oligonucleotides and RNA Interference. In Challenges in Delivery of Therapeutic Genomics and Proteomics; Elsevier, 2011; pp 325–386. https://doi.org/10.1016/B978-0-12-384964-9.00007-4.
[32] Jason, T. L. H.; Koropatnick, J.; Berg, R. W. Toxicology of Antisense Therapeutics. Toxicol Appl Pharmacol 2004, 201 (1), 66–83. https://doi.org/10.1016/j.taap.2004.04.017.
[33] Sepp-Lorenzino, L.; Ruddy, M. Challenges and Opportunities for Local and Systemic Delivery of SiRNA and Antisense Oligonucleotides. Clin Pharmacol Ther 2008, 84 (5), 628–632. https://doi.org/10.1038/clpt.2008.174.
[34] Roberts, T. C.; Langer, R.; Wood, M. J. A. Advances in Oligonucleotide Drug Delivery. Nature Reviews Drug Discovery. Nature Research October 1, 2020, pp 673–694. https://doi.org/10.1038/s41573-020-0075-7.
[35] Lin, C.-Y.; Huang, Z.; Jaremko, W.; Niu, L. High-Performance Liquid Chromatography Purification of Chemically Modified RNA Aptamers. Anal Biochem 2014, 449, 106–108. https://doi.org/10.1016/j.ab.2013.12.022.
[36] Zhang, Q.; Lv, H.; Wang, L.; Chen, M.; Li, F.; Liang, C.; Yu, Y.; Jiang, F.; Lu, A.; Zhang, G. Recent Methods for Purification and Structure Determination of Oligonucleotides. Int J Mol Sci 2016, 17 (12), 2134. https://doi.org/10.3390/ijms17122134.
[37] Gelfi, C.; Perego, M.; Morelli, S.; Nicolin, A.; Righetti2, P. G. Analysis of Antisense Oligonucleotides by Capillary Electrophoresis, Gel-Slab Electrophoresis, and HPLC: A Comparison; Mary Ann Liebert, Inc, 1996; Vol. 6. www.liebertpub.com.
[38] Hayashida, M.; Suzuki, R.; Horie, S.; Masuda, J.; Yamaguchi, T.; Obika, S. Applicability of Supercritical Fluid Chromatography for Oligonucleotide Analysis: A Proof-of-Concept Study. J Chromatogr A 2023, 1708. https://doi.org/10.1016/j.chroma.2023.464333.
[39] Lardeux, H.; Goyon, A.; Zhang, K.; Nguyen, J. M.; Lauber, M. A.; Guillarme, D.; D’Atri, V. The Impact of Low Adsorption Surfaces for the Analysis of DNA and RNA Oligonucleotides. J Chromatogr A 2022, 1677. https://doi.org/10.1016/j.chroma.2022.463324.
[40] Shibayama, S.; Sakamaki, H.; Yamazaki, T.; Takatsu, A. Metal Free Columns for Determination of Deoxynucleotide Monophosphate by Liquid Chromatography/Mass Spectrometry and Application to Oligonucleotide. J Chromatogr A 2015, 1406, 210–214. https://doi.org/10.1016/j.chroma.2015.06.033.
[41] Chornyy, S.; Parkhomenko, Y.; Chorna, N. Thiamine Antagonists Trigger P53-Dependent Apoptosis in Differentiated SH-SY5Y Cells. Sci Rep 2017, 7 (1). https://doi.org/10.1038/s41598-017-10878-x.
[42] Chen, J.; Stubbe, J. Synthesis and Characterization of Oligonucleotides Containing a 4‘-Keto Abasic Site. Biochemistry 2004, 43 (18), 5278–5286. https://doi.org/10.1021/bi0495376.
[43] LaFrancois, C. J.; Yu, K.; Sowers, L. C. Quantification of 5-(Hydroxymethyl)Uracil in DNA by Gas Chromatography/Mass Spectrometry: Problems and Solutions. Chem Res Toxicol 1998, 11 (7), 786–793. https://doi.org/10.1021/tx970233c.
[44] Gu, F.; Stillwell, W. G.; Wishnok, J. S.; Shallop, A. J.; Jones, R. A.; Tannenbaum, S. R. Peroxynitrite-Induced Reactions of Synthetic Oligo 2‘-Deoxynucleotides and DNA Containing Guanine: Formation and Stability of a 5-Guanidino-4-Nitroimidazole Lesion. Biochemistry 2002, 41 (23), 7508–7518. https://doi.org/10.1021/bi020148q.
[45] Liu, A.; Cheng, M.; Zhou, Y.; Deng, P. Bioanalysis of Oligonucleotide by LC–MS: Effects of Ion Pairing Regents and Recent Advances in Ion-Pairing-Free Analytical Strategies. Int J Mol Sci 2022, 23 (24), 15474. https://doi.org/10.3390/ijms232415474.
[46] Cui, Z.; Theruvathu, J. A.; Farrel, A.; Burdzy, A.; Sowers, L. C. Characterization of Synthetic Oligonucleotides Containing Biologically Important Modified Bases by Matrix-Assisted Laser Desorption/Ionization Time-of-Flight Mass Spectrometry. Anal Biochem 2008, 379 (2), 196–207. https://doi.org/10.1016/j.ab.2008.04.031.
[47] Hagan, N. A.; Smith, C. A.; Antoine, M. D.; Lin, J. S.; Feldman, A. B.; Demirev, P. A. Enhanced In-Source Fragmentation in MALDI-TOF-MS of Oligonucleotides Using 1,5-Diaminonapthalene. J Am Soc Mass Spectrom 2012, 23 (4), 773–777. https://doi.org/10.1007/s13361-011-0333-3.